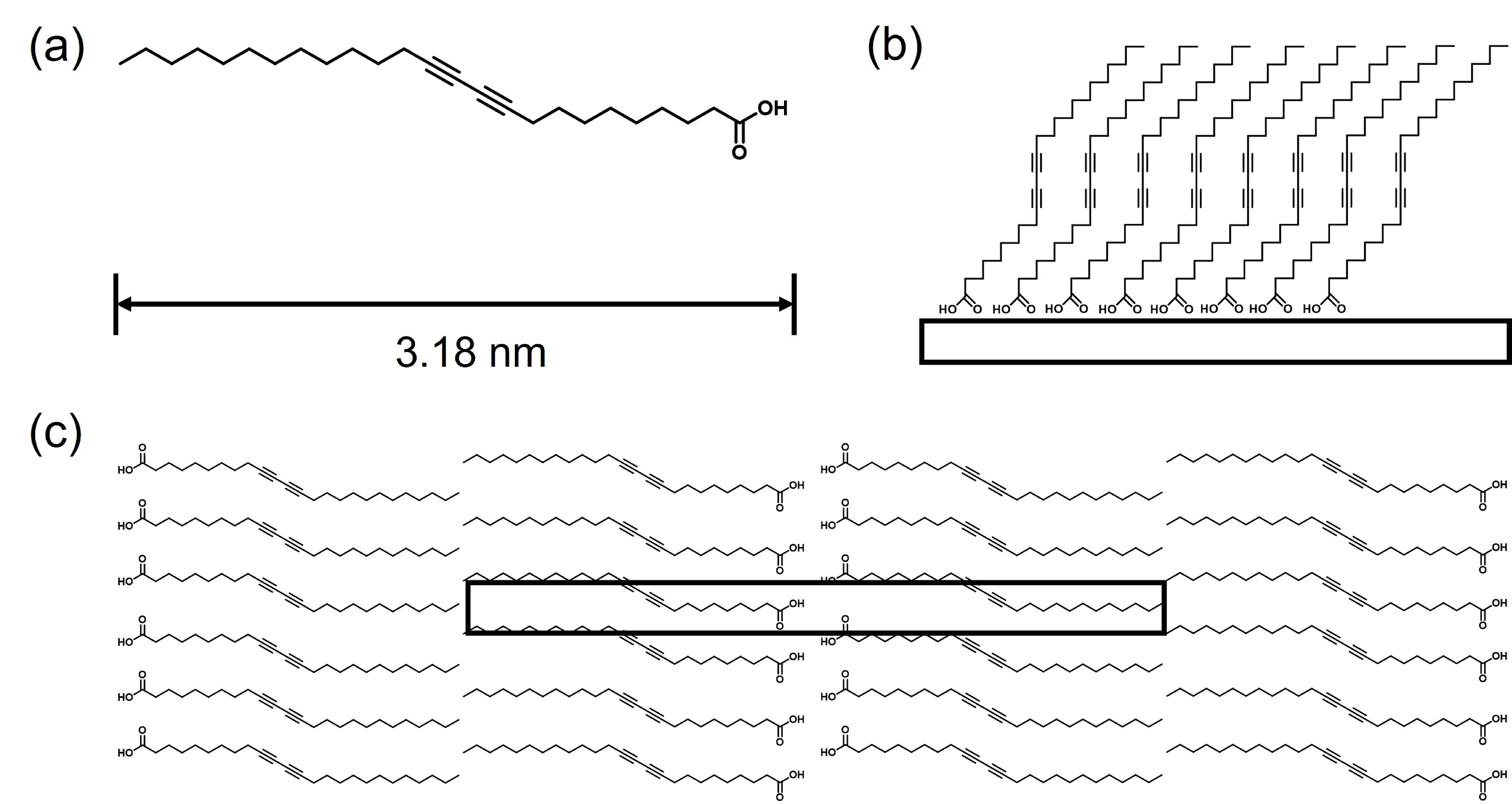
Self-assembly films have demonstrated an efficient method to functionalize the surfaces of variously different materials. In this work, we preliminarily explored the modification effect of 10,12-pentacosadiynoic acid (PCDA) on the optical properties of monolayer molybdenum disulfide (MoS2) grown on a rutile titanium dioxide (r-TiO2) (110) single crystal surface. Atomic force microscopy (AFM) characterizations directly revealed that the PCDA molecules self-assemble into the same lamella structure as on pure MoS2, which can be further polymerized into conductive polydiacetylene (PDA) chains under ultraviolet light (UV) irradiation. Detailed photoluminescence (PL) measurements observed clearly increased luminescence of negative trions (A−) yet decreased total intensities for MoS2 upon adding the PCDA assembly, which is further enhanced after stimulating its polymerization. These results indicate that the PCDA assembly and its polymerization have different electron donability to MoS2, which hence provides a deepened understanding of the interfacial interactions within a multicomponent system. Our work also demonstrates the self-assembly of films as a versatile strategy to tune the electronic/optical properties of hybridized two-dimensional materials.
Self-assembly films of PCDA and its in situ photopolymerization donate different amount of electrons to the MoS2/TiO2 surface and thus modulate the photoluminescence of the latter.
Figure 2. Characterization of the as-prepared MoS2/TiO2 substrate. (a) AFM image of an atomically flat TiO2(110) surface. The inset in the upper right corner shows the profile taken along the black arrow. (b) AFM image of a triangular MoS2 flake on the MoS2/TiO2 surface. The upper right inset shows the height profile measured across the MoS2 boundary along the black arrow. (c) Typical SEM image of the as-prepared MoS2/TiO2 surface. (d) Raman spectrum of MoS2 transferred from TiO2 onto a SiO2/Si substrate. (e, f) High-resolution XPS spectra of Mo 3d and S 2p on MoS2/TiO2.
Figure 3. (a) AFM and (b) the corresponding STM characterizations of the self-assembly film of PCDA formed on MoS2. Four PCDA molecular models are overlapped over the STM image. Note that although the film coverage slightly surpasses that of the monolayer, STM can only observe the structure of the bottom layer. (c, d) are the section profiles taken along the black and blue lines, respectively. The measured distances correspond to ten and double times the periodicities in these directions.
Figure 4. AFM characterization of the assembly structure of PCDA on the MoS2/TiO2 surface. (a) Large-area topographic image showing the PCDA aggregates formed on the MoS2/TiO2 surface. (b) Zoomed-in topographic image focusing on the MoS2/TiO2 region covered by PCDA film. (c) Zoomed-in phase image showing the resolved assembly structure of PCDA on MoS2/TiO2. (d) Section profile taken along the white arrow in (c), which measures the periodicity of the PCDA assembly as ~6.9 nm.
Figure 5. (a) AFM topography and (b) the corresponding phase images of the PCDA/MoS2/TiO2 surface after UV irradiation. The black and red ovals highlight the polymerized and unreacted PCDA assembly, respectively. (c) Section profiles taken along the black and red arrows in (a), showing the height difference between the reacted and unreacted PCDA film. Inserted are the simple schematics for the flat lying PCDA (lower) and the PDA polymer (upper), respectively.
[1] |
Li Y, Duerloo K A, Wauson K, et al. Structural semiconductor-to-semimetal phase transition in two-dimensional materials induced by electrostatic gating. Nat. Commun., 2016, 7: 10671. DOI: 10.1038/ncomms10671
|
[2] |
Liu E, Fu Y, Wang Y, et al. Integrated digital inverters based on two-dimensional anisotropic ReS2 field-effect transistors. Nat. Commun., 2015, 6: 6991. DOI: 10.1038/ncomms7991
|
[3] |
Iqbal M W, Iqbal M Z, Khan M F, et al. Tailoring the electrical and photo-electrical properties of a WS2 field effect transistor by selective n-type chemical doping. RSC Adv., 2016, 6 (29): 24675–24682. DOI: 10.1039/C6RA02390H
|
[4] |
Chen B, Meng Y H, Sha J W, et al. Preparation of MoS2/TiO2 based nanocomposites for photocatalysis and rechargeable batteries: progress, challenges, and perspective. Nanoscale, 2018, 10 (1): 34–68. DOI: 10.1039/C7NR07366F
|
[5] |
Li Y H, Cai C Z, Gu Y H, et al. Novel electronic properties of a new MoS2/TiO2 heterostructure and potential applications in solar cells and photocatalysis. Appl. Surf. Sci., 2017, 414: 34–40. DOI: 10.1016/j.apsusc.2017.04.001
|
[6] |
Tu W G, Li Y C, Kuai L B, et al. Construction of unique two-dimensional MoS2-TiO2 hybrid nanojunctions: MoS2 as a promising cost-effective cocatalyst toward improved photocatalytic reduction of CO2 to methanol. Nanoscale, 2017, 9 (26): 9065–9070. DOI: 10.1039/C7NR03238B
|
[7] |
Xiang M M, Huang C X, Xing Y, et al. Modulated photoluminescence of single-layer MoS2 on various rutile TiO2 surfaces: implications for photocatalytic applications. ACS Appl. Nano Mater., 2022, 5 (5): 7609–7618. DOI: 10.1021/acsanm.2c01754
|
[8] |
Liu H H, Li Y, Xiang M M, et al. Single layered MoS2 directly grown on rutile TiO2(110) for enhanced interfacial charge transfer. ACS Nano, 2019, 13 (5): 6083–6089. DOI: 10.1021/acsnano.9b02608
|
[9] |
Kagan C R, Mitzi D B, Dimitrakopoulos C D. Organic-inorganic hybrid materials as semiconducting channels in thin-film field-effect transistors. Science, 1999, 286 (5441): 945–947. DOI: 10.1126/science.286.5441.945
|
[10] |
Jariwala D, Howell S L, Chen K S, et al. Hybrid, gate-tunable, van der Waals p-n heterojunctions from pentacene and MoS2. Nano Lett., 2016, 16 (1): 497–503. DOI: 10.1021/acs.nanolett.5b04141
|
[11] |
Yu S H, Lee Y, Jang S K, et al. Dye-sensitized MoS2 photodetector with enhanced spectral photoresponse. ACS Nano, 2014, 8 (8): 8285–8291. DOI: 10.1021/nn502715h
|
[12] |
Yuan Y J, Lu H W, Ji Z G, et al. Enhanced visible-light-induced hydrogen evolution from water in a noble-metal-free system catalyzed by ZnTCPP-MoS2/TiO2 assembly. Chem. Eng. J., 2015, 275: 8–16. DOI: 10.1016/j.cej.2015.04.015
|
[13] |
Guo C, Xue J D, Cheng L X, et al. Two-dimensional self-assembly of diacetylenic acid derivatives and their light-induced polymerization on HOPG surfaces. Phys. Chem. Chem. Phys., 2017, 19 (24): 16213–16218. DOI: 10.1039/C7CP02337E
|
[14] |
Giridharagopal R, Kelly K F. Substrate-dependent properties of polydiacetylene nanowires on graphite and MoS2. ACS Nano, 2008, 2 (8): 1571–1580. DOI: 10.1021/nn800287x
|
[15] |
Okawa Y, Akai-Kasaya M, Kuwahara Y, et al. Controlled chain polymerisation and chemical soldering for single-molecule electronics. Nanoscale, 2012, 4 (10): 3013–3028. DOI: 10.1039/c2nr30245d
|
[16] |
Liu H H. Direct fabrication of 2D materials on oxide surface and investigation of the interface properties. Thesis. Hefei: University of Science and Technology of China, 2018. (in Chinese)
|
[17] |
Yamamoto Y, Nakajima K, Ohsawa T, et al. Preparation of atomically smooth TiO2 single crystal surfaces and their photochemical property. Jpn. J. Appl. Phys., 2005, 44 (17): L511–L514. DOI: 10.1143/JJAP.44.L511
|
[18] |
Wu J X, Liu J P, Cui J, et al. Dual-phase MoS2 as a high-performance sodium-ion battery anode. J. Mater. Chem. A, 2020, 8 (4): 2114–2122. DOI: 10.1039/C9TA11913B
|
[19] |
Lee C, Yan H, Brus L E, et al. Anomalous lattice vibrations of single-and few-layer MoS2. ACS Nano, 2010, 4 (5): 2695–2700. DOI: 10.1021/nn1003937
|
[20] |
Ho Y T, Ma C H, Luong T T, et al. Layered MoS2 grown on c-sapphire by pulsed laser deposition. Phys. Status Solidi RRL, 2015, 9 (3): 187–191. DOI: 10.1002/pssr.201409561
|
[21] |
Ahn C, Lee J, Kim H U, et al. Low-temperature synthesis of large-scale molybdenum disulfide thin films directly on a plastic substrate using plasma-enhanced chemical vapor deposition. Adv. Mater., 2015, 27 (35): 5223–5229. DOI: 10.1002/adma.201501678
|
[22] |
Hao S, Yang B C, Gao Y L. Unravelling merging behaviors and electrostatic properties of CVD-grown monolayer MoS2 domains. J. Chem. Phys., 2016, 145 (8): 084704. DOI: 10.1063/1.4961509
|
[23] |
Mandal S K, Okawa Y, Hasegawa T, et al. Rate-determining factors in the chain polymerization of molecules initiated by local single-molecule excitation. ACS Nano, 2011, 5 (4): 2779–2786. DOI: 10.1021/nn103231j
|
[24] |
Verveniotis E, Okawa Y, Makarova M V, et al. Self-assembling diacetylene molecules on atomically flat insulators. Phys. Chem. Chem. Phys., 2016, 18: 31600–31605. DOI: 10.1039/C6CP06749B
|
[25] |
Splendiani A, Sun L, Zhang Y, et al. Emerging photoluminescence in monolayer MoS2. Nano Lett., 2010, 10 (4): 1271–1275. DOI: 10.1021/nl903868w
|
[26] |
Yuan Y J, Lu H W, Yu Z T, et al. Noble-metal-free molybdenum disulfide cocatalyst for photocatalytic hydrogen production. ChemSusChem, 2015, 8 (24): 4113–4127. DOI: 10.1002/cssc.201501203
|
[27] |
van der Zande A M, Huang P Y, Chenet D A, et al. Grains and grain boundaries in highly crystalline monolayer molybdenum disulphide. Nat. Mater., 2013, 12 (6): 554–561. DOI: 10.1038/nmat3633
|
[28] |
Mak K F, He K, Lee C, et al. Tightly bound trions in monolayer MoS2. Nat. Mater., 2013, 12 (3): 207–211. DOI: 10.1038/nmat3505
|